Bill David
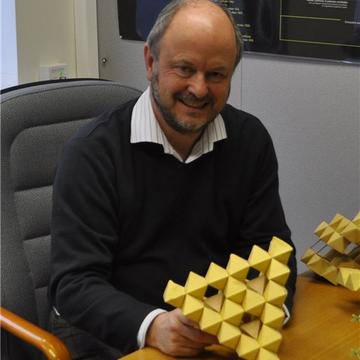
Professor Bill David FRS
Professor of Inorganic Chemistry
Our research spans the fields of energy materials chemistry and the atomic, molecular and nano-structure of materials. Our energy materials studies centre on the discovery and characterisation of novel chemical energy storage systems and we have a particular focus in developing hydrogen and ammonia as energy vectors. We use neutron and synchrotron X-ray diffraction combined with computational modelling as our principal techniques for the structural elucidation of materials. In the area of energy materials, our studies have included in-situ neutron powder diffraction measurements combined with gravimetric analysis for the study of reversible lightweight hydrogen storage materials and mass spectrometry for the investigation of novel ammonia decomposition materials.
Novel energy materials systems | permuting the odds
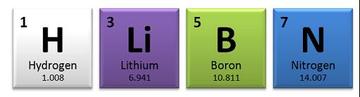
Our search for lightweight hydrogen storage systems has uncovered a broad range of novel compounds. Indeed, permuting just the first four odd elements – hydrogen, lithium, boron and nitrogen – leads to a large set of diverse materials that include complex salts, metals and molecular crystals. The properties of these compounds range from solid and liquid, hydrogen and ammonia storage materials through to superionic conductors, solid electrolytes and lightweight metals.
Hydrogen storage materials
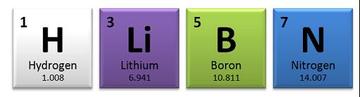
Effective solid state hydrogen storage remains an elusive goal in the transition towards sustainable, hydrogen-based transportation. Although many systems have been investigated, there have been few significant advances towards a truly practical system. Most complex metal hydrides with sufficient volumetric and gravimetric hydrogen density do not have an acceptably low hydrogen release temperature and are generally poorly reversible. Given the lack of success in the development of commercially viable solid state hydrogen storage materials, we believe a realigned focus on the mechanisms of hydrogen storage and release in complex hydride materials is essential, particularly amongst canonical systems that already display desirable characteristics. The lithium amide–lithium hydride composite system (Li–N–H) was first highlighted as a potential hydrogen store over a decade ago and remains a subject of interest because of its facile reversibility.
Our latest in-situ synchrotron powder X-ray diffraction measurements indicate that non-stoichiometric intermediates of the form Li1+xNH2-x are integral to the mechanism of both hydrogenation and dehydrogenation of the Li–N–H system. These observations are consistent with our proposed Frenkel defect model[4] for these reactions, underlining the importance of ionic mobility for the facile reversibility of this system.
While showing that non-stoichiometric Li1+xNH2-x phases are integral to the reaction mechanism of the Li–N–H system, we also observed the thermodynamic instability of intermediate stoichiometry components (BLi1.5NH1.5) towards ordering/phase separation into LiNH2 and Li2NH. This effect highlights the importance of in-situ measurements and may be explained by the different barriers to diffusion in the end member phases relative to the intermediate species. These results indicate potential avenues for (i) reducing capacity loss in this system and (ii) exploring the nature of the temperature limitation on reversible hydrogen capacity.
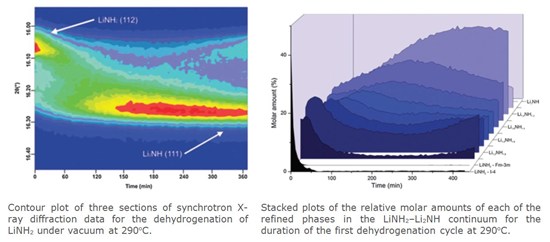
In-operando diffraction studies of metal hydrides for hydrogen fuel-cell electric vehicles
As a possible hydrogen storage system for fuel cell electric vehicles (FCEV), the new high pressure metal hydride (MH) tank system has been reported. [ 8 ] Ti-V-Cr-Mo alloys of body-centred cubic (bcc) structure, which has high reversible hydrogen capacity (2.4 mass% H 2) and high dissociation pressure (2.3 MPa at 298 K), have been developed for this MH tank system. So far, hydrogen storage properties of bcc alloys have been widely studied and it is known that these alloys have the highest reversible hydrogen capacity among current transition metal hydrides, but the hydrogen storage capacity decreases during the absorption and desorption cycle. In this communication, we report neutron diffraction studies of hydrogen absorption and desorption in a prototypic metal hydride under realistic operating conditions. These in operando neutron diffraction measurements mimic the hydrogen cycling behaviour in the current generation of FCEV. We clarify the detailed behaviour of hydrogen within the alloy crystal structure on absorption and desorption and identify the principal reasons for the degradation of hydrogen storage performance. Our results explain the nature of hydrogen absorption and desorption in the prototypic Ti-V-Cr-Mo transition metal hydride system and also the capacity limitations that develop on prolonged cycling of these materials. We conclude that even with this generation of transition metal hydride the degree of hydrogen sequestration on cycling may be eliminated, which in turn will lead to a higher usable gravimetric capacity. Further optimised alloy systems may reach storage capacities that will approach near-term transportation requirements for FCEV.
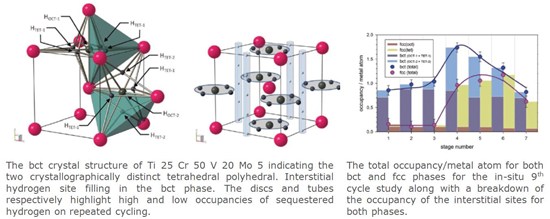
Ammonia as a future energy vector
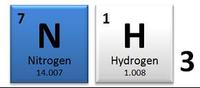
The big advantage of ammonia is that it can be carbon-free both in terms of its synthesis simply from air and water and also in terms of it combustion products – again simply air and water.
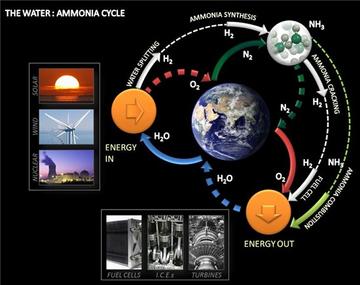
Ammonia can be combusted in an internal combustion engine (with about half the volumetric energy density of petrol), or used directly in high temperature fuel cells or decomposed to release its stored hydrogen in alkaline fuel cell. Applications range from transportation to long-term energy storage that enable a balanced supply of energy from intermittent renewables.
Given this potential, it begs the question why ammonia has been largely overlooked as a serious contender for the storage and distribution of hydrogen? There are two central reasons: safety, and the ability to extract its stored hydrogen. We conduct research with the aim of overcoming these barriers and enabling the use of ammonia as a sustainable fuel.
Novel ammonia decomposition processes
Traditional catalytic cracking of ammonia to nitrogen and hydrogen is only achieved at high temperatures, with expensive metal catalysts and with poor kinetics. We have recently demonstrated that light metal amides can also be used as catalysts for the decomposition of ammonia, showing superior high-conversion performance to even the most active metal catalyst, ruthenium. Our experiments indicate that sodium amide decomposes ammonia by two concurrent, stoichiometric chemical reactions, the synthesis and subsequent decomposition of the amide via the metal.
Na + NH3 → NaNH2 + ½ H2
NaNH2 → Na + ½ N2 + H2
This type of reaction mechanism represents a significant departure from traditional surface catalysis, and uses materials which are significantly cheaper and more abundant than transition metal catalyst systems.
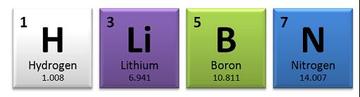
One of the principal issues about the widespread use of ammonia is concern about safety. Ammonia is a toxic chemical and, thus, efforts to safeguard against its exposure to people and the natural environment are an important component of facilitating its use as an energy vector. The high toxicity level of ammonia is primarily a consequence of its high vapour pressure. One option for removing ammonia from gas streams, or, indeed, for its storage, is to use solid-state ammine complexes, such as magnesium chloride hexa-ammoniate (MgCl2(NH3)6). These compounds reduce the vapour pressure of the ammonia to millibar levels, and in doing so can effectively render the ammonia non-toxic. Many also have an ammonia density similar to liquid ammonia, making them efficient methods of storing ammonia.
Structural materials science – redefining crystallography!
The extent to which crystallography is defined and limited by the word from which it is derived – crystal – is a topical point of current discussion but it is not just a modern debate.
The Oxford Dictionary defines crystallography to be the “the branch of science concerned with the structure and properties of crystals” while other definitions are even more restrictive. A browse through Wikipedia suggests that “crystallography is the experimental science of determining the arrangement of atoms in the crystalline solids”.
And yet, crystallography was a science well before the nature of the arrangements of atoms was known. The meaning of crystallography evolved after the discovery of X-ray crystallography and it deserves to evolve past the limitation imposed by ordered crystalline matter.
Science is littered with words whose meanings have broadened from their original, more narrow definitions. Take ferromagnetism for example. Not all ferromagnets contain iron and derivative words, such as antiferromagnetism and ferrimagnetism, have been defined to describe other forms of magnetism. And, indeed, other derived words that include ferroics, multiferroics, ferroelectricity and ferroelasticity have left magnetism far behind and generally have very little to do with iron. Words move on in science!
In their excellent 1973 essay entitled ”Structuration”, Alan Mackay and John Finney contended that
“the subject matter of crystallography is really structure (at various levels) and that the concern with crystals is only incidental.”
They further noted that “Crystals are exceptional” – in both senses of the word.
“Considered among other structures of comparable size, crystals are seen to be quite exceptional in that a single set of rules for assembly carry the structure from the level of the immediate surroundings of single atoms (5 A) to that of large crystals (10 cm). In terms which we will define below, the span of the assembly rule is exceptionally large. It follows that the structure - the position of every atom - of a large crystal can be described by very few parameters. The arrangement of all the atoms in a large crystal of quartz can be described with some 10-20 parameters, while the arrangement of the similar atoms in a physically and chemically rather similar piece of vitreous silica cannot yet be described with comparable accuracy. A similar number of parameters should characterize the structure of vitreous silica with similar precision. The problem is how to select the appropriate parameters. In the case of the crystal it is clearly the symmetry which enables us to do this.”
Perhaps the most significance challenge of understanding the non-crystalline and almost crystalline state is articulated above.
A similar number of parameters should characterize the structure of vitreous silica with similar precision. The problem is how to select the appropriate parameters.
This is not just a great structural challenge but an essential part of our understanding of the modern materials world. Perfect crystalline order is the extreme exception. Mackay and Finney go further and quote J D Bernal’s aphorism that “Crystallisation is death”!
From batteries and fuel cells through to the drugs that we ingest, the structure of our modern world from the angstrom to the micron and beyond may have a template of crystalline order but it is only fully defined when imperfections, heterogeneities, interfaces and the absence of long-range atomic order are taken into consideration. Much of our research is concerned with unravelling the structural complexity of materials and their behaviour under real-life conditions.
Superhard carbon | mesoscale rules!
One of the most remarkable contrasts that highlights the crucial importance of chemical bonding is catalogue of differences between the two principal allotropes of carbon, diamond and graphite. The most well-known of these is the difference in hardness. Diamond is a remarkably strong material while graphite is one of the softest substances known and only 10.53o separates the angles for sp2 and sp3 bonding. With the discovery of graphene, things have become a bit more complicated – graphene is harder even than diamond though the latter does have a greater tensile strength.
In 2010, the carbon story got even more convoluted with the discovery of carbon microspheres, made from plastic bags, which were as hard as diamond but, to all intents and purposes, appeared to be graphitic at an atomic level. These carbon microspheres also turn to be excellent lithium anode materials. We have halfway solved the riddle using a combination of electron microscopy and total neutron scattering measurements. Autogenic synthesis at 700oC produces soft microspheres composed of ~2nm. graphene-like flakes terminated with hydrogen bonds. Further firing at 2800oC produces the superhard carbon spheres that are excellent lithium anode materials. The hardness and lithium insertion are both associated with radially directed multiwall nanotube although the precise nature of these features is not yet known. It is the mesoscale structure that principally determines the properties of these amazing carbon microspheres. Our current challenge is to fit our total scattering neutron diffraction data to replicate in detail the pair distribution function up to 100Å with a structure that is consistent with the electron microscopy data.
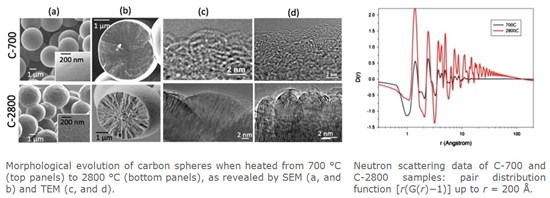
Structure determination from powder diffraction data
DASH
DASH is an interactive and accurate package for structure solution from powder diffraction data developed by STFC and the Cambridge Crystallography Data Centre (CCDC). In 1998, its first significant success was the determination of three complex molecular structures: capsaicin (I), thiothixene (II) and promazine hydrochloride (III). We are currently developing further extensions to DASH that will enable us to identify fragments and determine crystal structures when the correct molecular structure has not been fully determined.
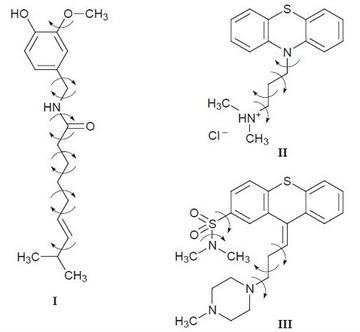
On the verge of crystallinity
Understanding and controlling the crystallisation of small molecular structures is a key challenge in organic chemistry and particularly in the pharmaceutical sciences. There are multiple parameters that determine crystallisation and many attempts, with varying success, have been made to develop a rational chemical approach to the understanding of crystallisation. Following a epidemiological approach based on machine-learning algorithms it has been possible to develop predictive criteria to determine the propensity for small organic molecules to form well-ordered crystalline phases.
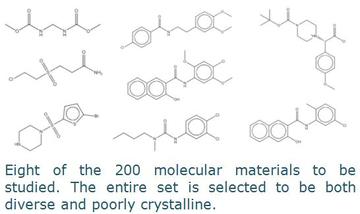
Using these predictive criteria, we have selected a set of organic molecular materials of unknown structure. A survey of microstructure determined from diffraction scans of all samples will be used to test the predictive accuracy of our model. We will determine the crystal structures of all the materials that show modest crystalline order and understand the factors that hinder the formation of large scale crystalline domains in these materials. The library of poorly crystalline datasets will provide a foundation for further development of structure solution methods for difficult-to-solve crystalline materials in DASH.
I read Physics at St. Catherine’s and stayed on at College to complete my DPhil in Physics at the Clarendon Laboratory. I then moved the short distance from the Clarendon to the Inorganic Chemistry Laboratory to take up a post-doctoral research position with Professor John Goodenough (previously Head of Inorganic Chemistry and now an Honorary Fellow of the College) working on some of the earliest lithium battery research. In 1983, I moved to the Rutherford Appleton Laboratory to help set up the diffraction facilities at what was to become the ISIS spallation neutron source. I returned to Oxford in 2006 as a Visiting Professor in Inorganic Chemistry and was elected to a Fellowship by Special Election in Physicss at the College. In October 2013, I was appointed to the joint position of Professor of Chemistry in Oxford and STFC Senior Fellow at ISIS.
Contact
bill.david@chem.ox.ac.uk
01865 272689
College
Please note that Prof. David is not recruiting graduate students or postdoctoral researchers.